The fastest current switching in a conductor — published in Nature
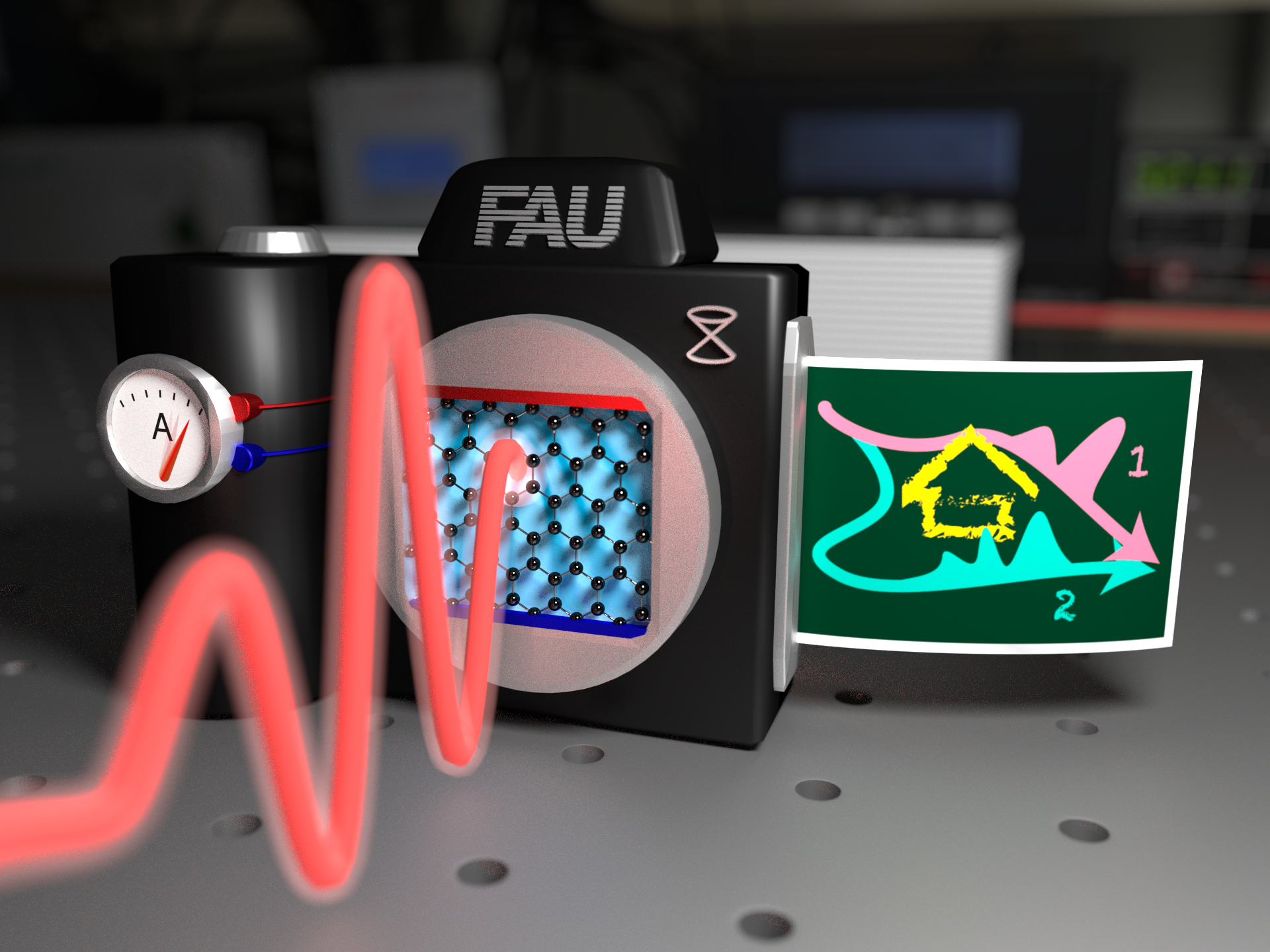
“How fast can one turn on a current?” is a fundamentally important question behind boosting up the speed of modern electronics, since the data and signals are transferred via the flow of electrons. In joint work with the group of Prof. Heiko B. Weber of FAU’s Applied Physics Chair, we have opened up a new channel for achieving ultrafast turning-on of currents in graphene, an exotic conducting material, on the timescale of a single femtosecond (1 femtosecond is a billionth of a millionth of a second).
When light detectors such as camera sensors or solar cells are illuminated with light, electrons in these materials are excited from the valence band to the conduction band, resulting in the generation of photocurrents. The timescale of this usual photocurrent generation is governed by the duration of the laser pulse because the light absorption occurs over the whole pulse duration. However, when intense laser pulses are employed, a significant population transfer from the valence to the conduction band may occur within a fraction of the laser pulse duration, in fact even within a fraction of a single optical cycle. Usually, a laser pulse consists of dozens to hundreds or thousands of optical cycles. In our case, we worked with extremely short optical pulses, consisting of just two oscillations. This short pulse duration and the large field strength allowed us to observe a transition to the so-called strong-field regime, which occurs when the strength of the light-matter interaction overtakes the photon energy.
This light-field-driven population transfer – so the field-driven excitation of electrons from the ground to an excited state – has been demonstrated in gaseous media and insulating materials, but hitherto not in a conducting materials. A challenge for conducting materials has been found in the fact that ordinary metals reflect light, and thus strong optical fields cannot be applied to the electrons inside of metals. Graphene, an atomically thin layer of carbon atoms, allows us to overcome this difficulty – it is so thin that the light can directly affect electrons in graphene while the light propagates through it.
We test the possibility to control currents in graphene with the light-field-driven process by illuminating graphene with linearly polarized few-cycle laser pulses with a controlled carrier-envelope phase (CEP), which is a key parameter to determine the electric field waveform of the pulse. We have found that the current generated in unbiased graphene is sensitive to the CEP. Furthermore, this CEP-dependent current changes its direction when we increase the laser intensity. The field strength of 2 V/nm at which this striking current direction reversal takes place matches our theoretical prediction, underscoring that the electron excitation process turns into the strong-field regime.
Furthermore, we have found out that in the strong-field regime the electron dynamics are well described as repeated Landau-Zener transitions: In the reciprocal space, the momentum of an electron changes due to the acceleration by the optical field. When the electron comes nearby the Dirac point of graphene’s band structure, at which the conduction and valence band touch each other, the electron’s wave function is coherently split into the superposition of the two band states. After a half optical cycle, these fractions of electron wave functions meet again, and depending on the phase relation between them, electrons are further excited to the conduction band or return to the valence band. The CEP of the laser pulse determines the phase relation governing this interference, known as Landau-Zener-Stückelberg (LZS) interference, and thus determines the direction of the current. Even furthermore, we can switch off the influence of this LZS interference by tuning the polarization state of light. Namely with circularly polarized light, the current does not change its direction as a function of the laser intensity, which is fully supported by our numerical simulations.
These results showcase an important step towards merging two major branches of modern technologies, electronics and optics, onto one and the same platform.
Our contribution has just appeared in Nature (published online on 25th Sep. 2017). The FAU issued a press release that can be found here.